Accepted 19 May 2020
Available Online 8 June 2020
- DOI
- https://doi.org/10.2991/mathi.k.200521.001
- Keywords
- Electrospinning
multifluid electrospinning
bio-applications
core–shell nanofibers
Janus nanofibers - Abstract
Electrospinning is a potent “top-down” nanofabrication method and has been explored by many researchers in recent decades because it is an efficient, versatile, simple, and low-cost route to prepare nanofibers. Nanofiber membranes generated by electrospinning have been used in various fields including tissue engineering, wound dressing, biosensing, theranostics, and functional textiles. Here we summarize the development of multifluid electrospinning processes, including coaxial, Janus and triaxial electrospinning, and the applications of these techniques. Complex nanostructures prepared by multifluid electrospinning are considered. The key parameters that affect the electrospinning process are introduced, and a discussion of both equipment and solution parameters is presented.
- Copyright
- © 2020 The Authors. Published by Atlantis Press SARL.
- Open Access
- This is an open access article distributed under the CC BY-NC 4.0 license (http://creativecommons.org/licenses/by-nc/4.0/).
1. INTRODUCTION
Along with the booming of nature science, bioscience has been explored widely and developed fast. Along with the knowledge from macro to micro, and further deeper to nano, nanotechnology has been developed fast, such as electrospinning, which uses a “top-down” way to produce nanomaterials. Nanomaterials have gradually entered the lives of people. Nanomaterials, including nanofibers and nanoparticles, have been explored in myriad fields, such as drug delivery systems [1], sewage treatment [2,3], material reinforcing [4], tissue engineering [5], catalysis [6], sensors [7,8], filtration membranes [9,10], osmosis membranes [11,12], and electrolytes [13] inter alia.
Electrospinning is a membrane preparation technology which has developed rapidly in recent decades. As the sister technology of electrospraying [14–17], takes advantage of the interactions between working fluids and electrical fields. It is a facile, efficient, economical, and flexible route to prepare nanoscale fibers via a “top-down” approach. Four key components are required to implement this process as shown in Figure 1: (1) a high (kV) voltage generator [18]; (2) an infusion pump, which can dispense solutions or melts at a precisely controlled rate; (3) a metal needle or spinneret; (4) a collector.
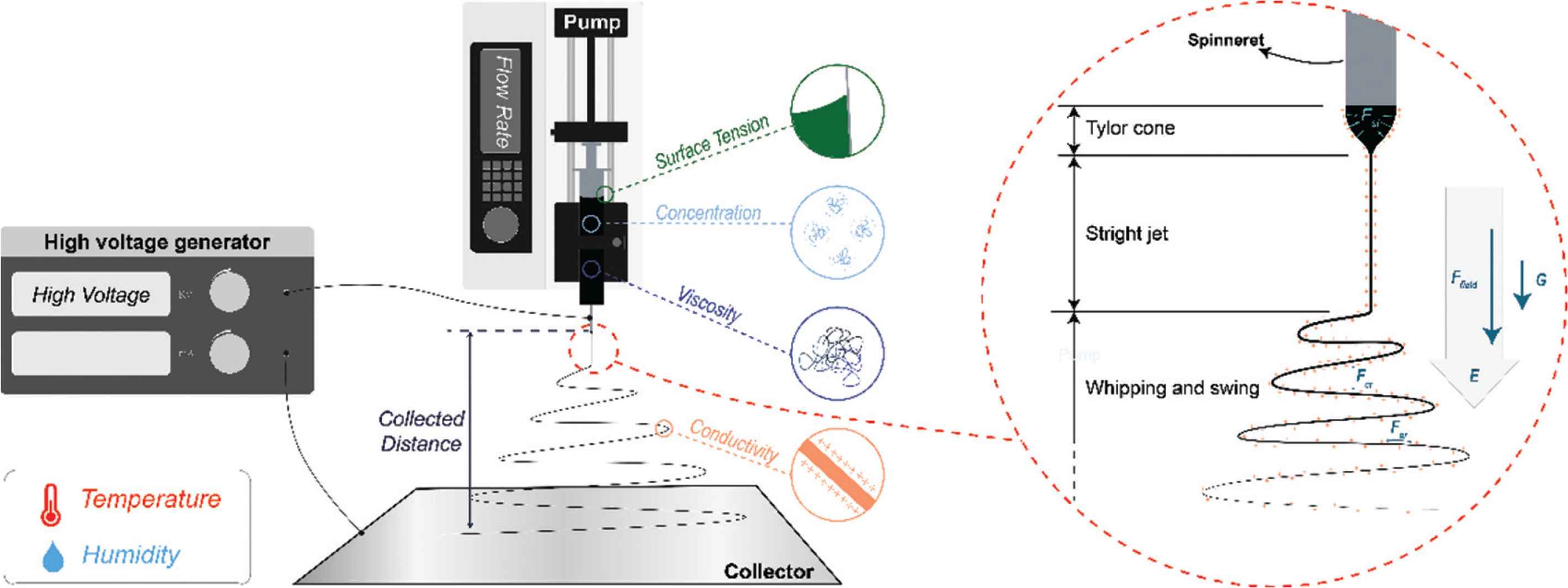
Key parameters affecting the electrospinning process, and three stages within electrospinning with interactions.
In electrospinning, a working fluid dispensed through the spinneret is stretched into nanoscale fibers by applying the high voltage electrostatic field (Ffield). The process can be divided into three steps as shown in Figure 1. First, the working fluid is extruded by the pump, and gathers on the tip of the spinneret. The droplet that would form in the absence of an electric field owing to surface tension is deformed into a conical shape (the Taylor cone) when a voltage is applied. This process is governed by a balance of gravity (G), electrostatic repulsion (Fer), and surface tension (Fst). Second, the jet would not be ejected with charges until the voltage exceeds a specific critical value, which means electrostatic repulsion exceeds surface tension, linear jet formed. Third, the linear jet could sustain a short time due to Rayleigh Taylor instability, and the interaction between charges along with jet and electrostatic field, therefore, whipping and swing as a consequence. Working fluid with charges in the electricity field will be elongated by electrostatic repulsion among like charges on the jet surface, Coulomb repulsion (Fcr) between layers, and gravity. Meanwhile, the solvent evaporates rapidly and cure into fibers in microscale or even nanoscale.
The development of electrospinning has taken place over a prolonged period of time, as shown in Figure 2. In the 16th century, William Gibert recorded and reported the phenomenon of electrostatic interactions with liquids. He found that when a moderate amount of charge exists on a liquid droplet’s surface, it deforms into a cone and then tiny drops are ejected from the tip. The droplet disintegrates into a mist under a suitable high electricity field. In 1885, the critical amount of charge required for droplet deformation was calculated by Lord Rayleigh, who proposed the first explanation of the electrospinning process [19].
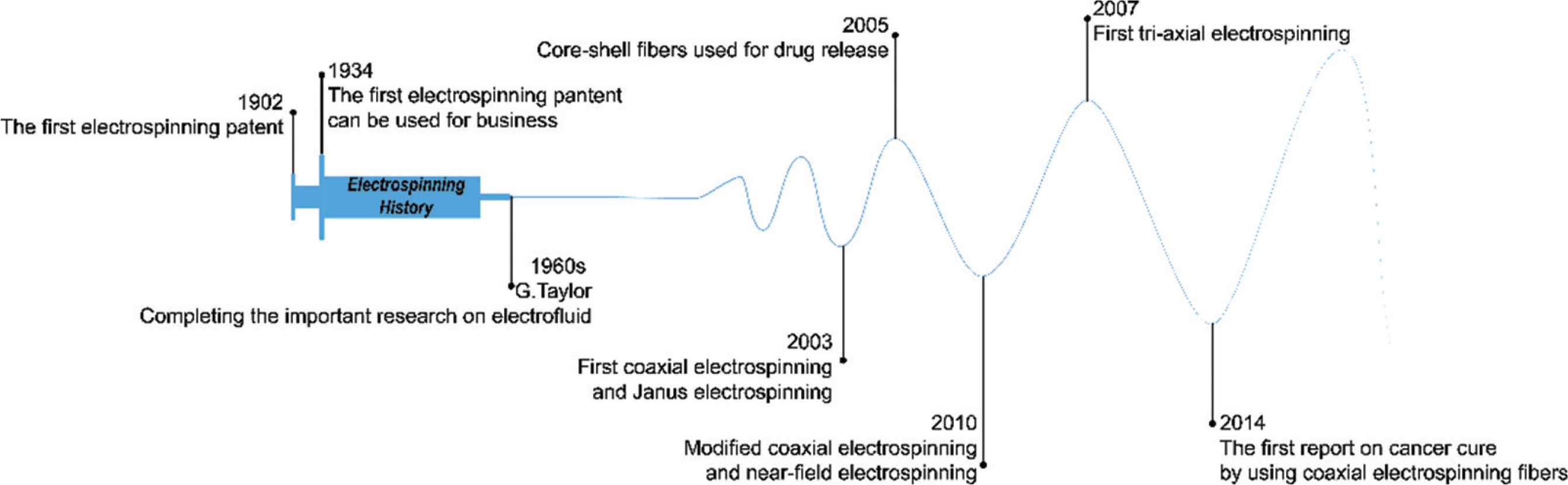
The development history of electrospinning.
In 1902, John Cooley was granted the first patent relating to electrospinning [20]. Formhals Anton applied for another patent in 1934, and this has been commercialized [21]. In the 1960s, Geoffrey Taylor systematically investigated the process of electrospinning [22,23], but after this time the technique fell out of favor. It was not until the 1990s that the group of Reneker conducted experiments to electrospin over 20 different polymers, and as a result were able to fabricate fibers on the nanoscale [24]. With the increasing interest in nanotechnology which the world has witnessed in recent decades, since this time the, electrospinning technology has developed rapidly. Sun et al. [25] built on the standard single-fluid electrospinning process and developed core-shell fibers by processing two liquids in coaxial electrospinning in 2003. In the same year, Gupta and Wilkes [26] realized a side-by-side electrospinning approach which could also process two liquids. Building on this, Lallave et al. [27] performed triaxial electrospinning (with three fluids), and Yu et al. [28] developed new coaxial electrospinning processes permitting a wider range of fluids to be processed [28] and Zheng et al. [29] started work on near-field electrospinning. Three years later, a four fluids electrospinning process was reported [30]. In parallel to the development of the electrospinning technology, the applications of electrospinning have also advanced, such as for tissue scaffolds [31], drug delivery [1] or cancer treatment [32].
2. MULTIFLUID ELECTROSPINNING PROCESSES
Multifluid electrospinning has been explored for a number of years, and used to prepare a range of complex structures [33]. This can have a range of additional functionalities or improved performance compared to monolithic fibers from mono-axial spinning. To date, the most widely studied processes are coaxial, side-by-side (or Janus), and triaxial electrospinning.
2.1. Coaxial Electrospinning
The coaxial spinneret was one of the four spinnerets developed by Cooley in 1902, and the other three are traditional single fluid spinneret, the air-assist spinneret, and a spinneret with rotating the valve distributor [20]. Coaxial electrospinning (or electrospray) has been further development among 2002 and 2003. Moreover, modified coaxial electrospinning technology vastly expands the solution, which can be adopted in electrospinning.
Coaxial electrospinning provides the opportunity to develop diverse nanostructures (see Figure 3a and 3b) with tunable functional performance. Coaxial electrospinning can be used to fabricate core-shell fibers (Figure 3a) [34,35], or hollow fibers (Figure 3b) [36–39]. Such core-shell structures can provide more functions. For example, heterojunction, which is a better way to transfer charge, can be formed between core and sheath [6,40,41]. For example, the sheath can be used to increase hydrophilicity, and the core to increase fiber strength. This has been found to produce materials which can be used for seawater desalination [42]. In addition, other properties of the core and sheath can also be implemented, such as concentration distribution [43], strength performance [44], or hydrophilic and hydrophobic properties [42].
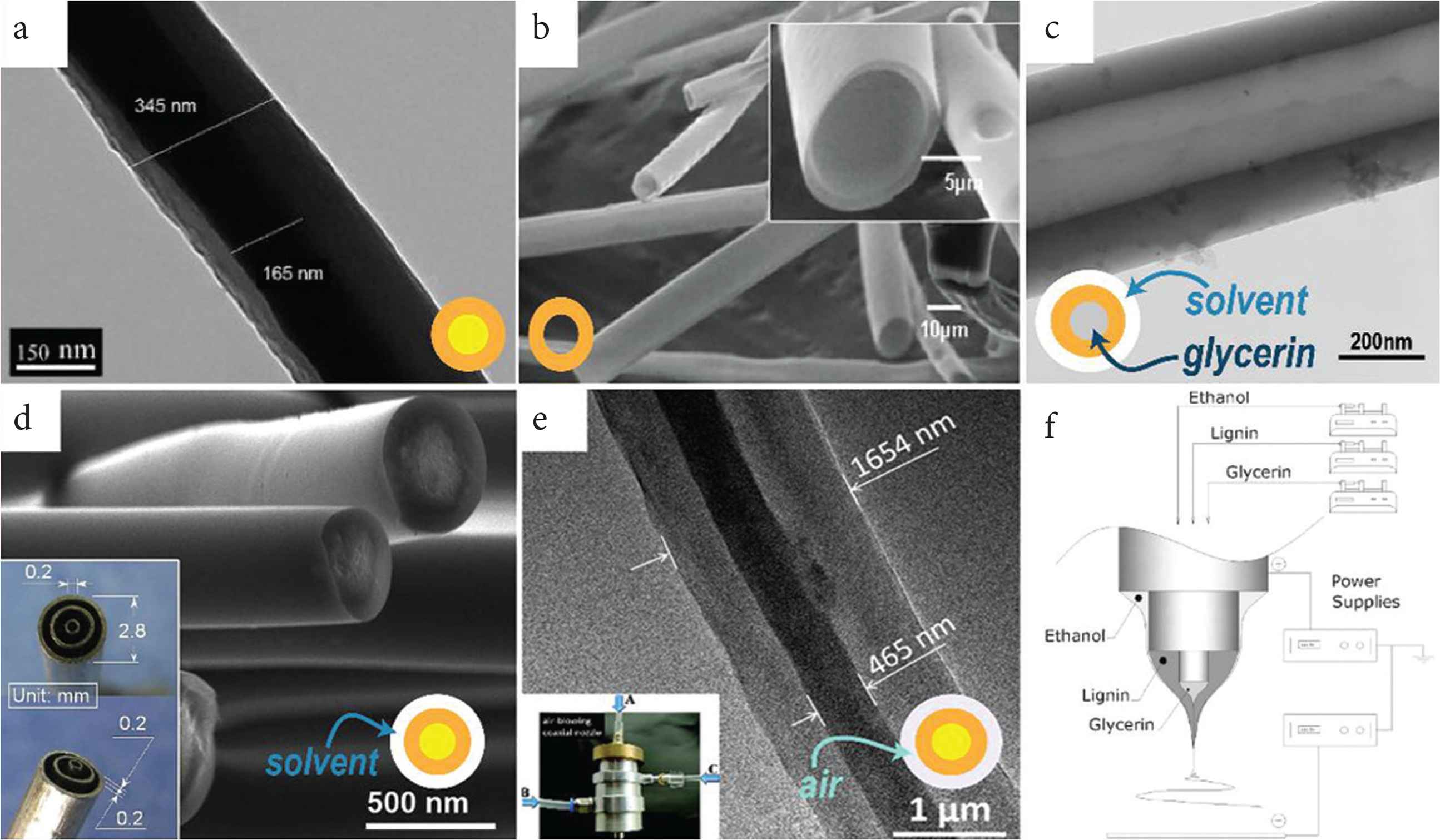
Core–shell and hollow fibers prepared by coaxial electrospinning. (a) Core–shell structure [Transmission Electron Microscopy (TEM) image]. (b) Hollow structure [Scanning Electron Microscopy (SEM) images]. Core–shell and hollow fibers prepared by tri-axial electrospinning. (c) single-wall hollow structure (TEM image). (d) Core–shell structure via modified tri-axial electrospinning (SEM image and the spinneret as inset) [45]. (e) Core–shell structure via air-blowing-assisted coaxial electrospinning [TEM image and picture for blow-assisted set: (A) inner capillary, (B) middle capillary, (C) outer bracket for air blow]. (f) It is the general set-up of (c) with different solutions in each layer.
Meantime, in modified coaxial electrospinning, first reported by Yu et al. [28], an unspinnable solution, which has too few chain entanglements to implement electrospinning process, could be used as the sheath solution. This could prevent the solvent rapidly evaporating (which is problematic because it might cause the fibers to have a rough surface). Furthermore, the working fluid can be more effectively well stretched in the electric field in the presence of a sheath solvent. As a result, the fibers produced tend to be finer. Using a modified coaxial electrospinning process with a pure solvent or dilute solution as the shell, problems which often arise with the formation of solid substances at the spinneret (and cause it to become blocked) and continuous electrospinning can be achieved [46,47].
2.2. Janus Electrospinning
The Janus structure was first achieved using electrospinning by Gupta and Wilkes [26]. The Janus structure can be a better choice than uniaxial electrospinning in some cases, because two kinds of materials that provide different functions will affect each other. The separation will let them perform well [48,49]. A fiber with two parts and can realize two or three functions those can be mutual independence, such as fluorescence and magnetic properties [48,50,51]. Fluorescence property are easily affected by magnetic substances, which will lower the fluorescence property if the two materials mixed in one fiber. And they also can work together, such as light absorption and hydrophilicity [52], adsorption property and photocatalytic performance [6].
Two kinds of spinnerets have been used to fabricate Janus nanofibers. One comprises cylinders arranged side by side; the other one is a small cylinder touch a bigger cylinder internally as Figure 4A(a and c) [53,54]. With the former spinneret, it is very hard to prepare Janus nanofibers, because as a result of Coulombic repulsion there is a tendency of the fluids to repel one another and split apart [55]. As a result, researchers have developed more spinneret designs such as that shown in Figure 4B(a–c) [56]. Moreover, the latter spinneret enlarges the contact area between the two working fluids, which means the viscous force is increased. Janus fibers can also be prepared smoothly and continuedly if there is a shell solution around the two side-by-side fluids [Figure 4A(b and d)]. Compared with Figure 4A(f) has few other finer fiber, Figure 4A(g) has a smoother morphology and clear Janus structure. Conjugate electrospinning process [Figure 4C(c)] can also prepare Janus nanofibers with good size contribution [Figure 4C(b)] and use a different method. Coulomb attraction plays a vital role in binding two fibers together, and then Janus fiber formed. For now, three forces can be used to conduct Janus electrospinning. They are Coulomb repulsion, Coulomb attraction, and viscous force, respectively. There may be other forces that could be used in electrospinning. And the combination of conjugate electrospinning and complex structure spinnerets could produce many possibilities.
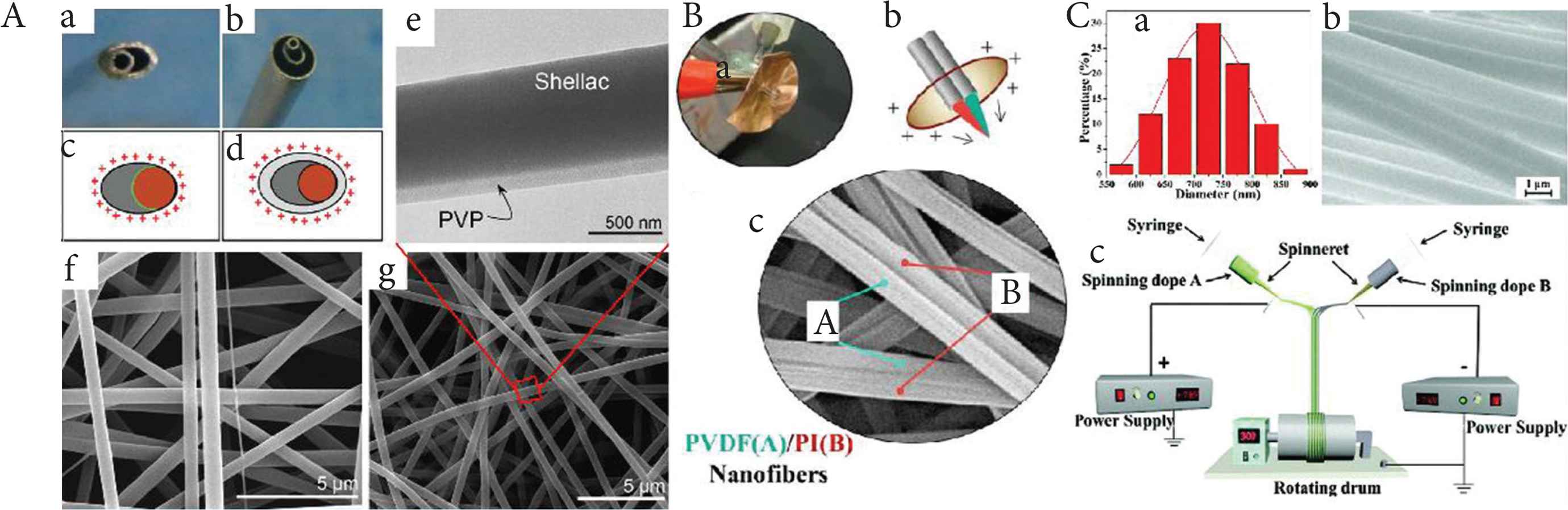
The influence of the spinneret on the structure of electrospun fibers. (A) Janus electrospinning: (a and b) pictures of the acentric and structured spinnerets; (c and d) schematics of the charged distribution and fluid contact interfaces; (e) Janus structures from structured spinneret F(TEM image); (f and g) fibers from acentric and structured spinnerets (SEM image) - Published by The Royal Society of Chemistry. (B) Side-by-side electrospinning: (a and b) picture and schematic of the specific spinnerets; (c) Janus structure nanofibers (SEM image). (C) Conjugate electrospinning: (a) diameter distribution of nanofibers in Janus Nanofiber Array Pellicle (JNAP); (b) SEM images of JNAP; (c) schematic diagram of the conjugate electrospinning - Published by The Royal Society of Chemistry.
2.3. Triaxial Electrospinning
Triaxial electrospinning uses three cylinders nested concentrically as the spinneret, which can be used for the electrospraying process as well, which uses unspinnable solutions to produce nanoparticles through same technalogy [57–59]. Here control of the operational parameters is particularly complicated because of the interaction between working fluids. Recently, triaxial electrospinning has begun to attract increasing attention; the recent reported on triaxial fibers are summarized in Table 1. Typical triaxial fibers are shown in Figure 5a.
Structure | Material | Application & purpose | References | ||
---|---|---|---|---|---|
Outer | Middle | Inner | |||
Triaxial nanofiber | Silica | Block copolymer | Silica | Exploring the self-assembly behavior of block copolymers in the constrained state | [60] |
Triaxial nanowire | Amorphous carbon | LiFePO4/Amorphous carbon | VGCF | Potential in Li-ion battery | [65] |
Nanowire-in-microtube structured core/shell fiber | Ti(OBu)4 solution | Paraffin oil emulsion | Ti(OBu)4 solution | Extra properties could be introduced due to the special structure | [61] |
Triaxial capsule | Poly(lactic-co-glycolic Acid) (PLGA) | Paclitaxel/poly (DL-Lactic Acid) | Doxorubicin/PLGA | Reducing initial burst release, managing capsule swelling and drug-diffusion times | [66] |
Triaxial nanofiber | Gelatin | Polycaprolactone (PCL) | Gelatin | Potential in biotechnology | [62] |
Triaxial fiber | PCL in TFE | PCL in CF:TFE (3:1) | Polyvinyl Pyrrolidone (PVP)/KAB dye | Enabling different drugs release profiles from a membrane | [67] |
Nanofibers store nanoparticles | Carbon | Silicon | Carbon | Potential in Li-ion batteries | [63] |
Three-layered triaxial fiber | PS | TPU | PS | Improving the mechanical properties | [68] |
Triaxial nanofiber | 4.17% Ketoprofen (KET)/Ethyl Cellulose (EC) | 17.86% KET/EC | 39.47% KET/EC | Precisely control drug release in zero-order profile | [69] |
Triaxial healing fiber | PMMA/PS/poly-(glycidyl methacrylate-co-styrene) | PAAm | Healing agent | Preserving the mechanical properties by self-repairing | [70] |
Multi-walled triaxial hollow fiber | PMMA | PAAm | - | Enhancing properties of composite materials | [4] |
PS | PAAm | ||||
Multilayered nanofiber | PCL | Polyvinyl Alcohol (PVA) | - | Exploring the effect of solvent mixture properties on the triaxial jet stability and its effect on mechanical property | [71] |
PCL | CA | ||||
CA | PCL | ||||
Core–shell fiber | Ethanol | lignin | glycerin | Hollow carbon fiber was prepared by triaxial electrospinning | [72] |
Triaxial fiber membrane | CA | PCL | Nisin | Long-term antimicrobial | [73] |
Triaxial fiber | PCL-GT | Hydrophilic Gelatin (GT) | PCL-GT | Precisely controlling drug release | [74] |
Triaxial fiber | PMMA | PAAm | LY 564 resin and XB 3458 hardener | Monitoring the interface and bulk self-healing | [75] |
Coaxial nanofiber | Solvent mixture | CA | Ferulic Acid (FA) | Depot drug within the fiber for sustained-release | [76] |
Triaxial nanofiber | DOX and PTX@ g-C3N4/PLA/CS | PLA/CS | CS/PVA/5-FU | Three drugs-controlled release simultaneously used for breast cancer | [77] |
Core–shell fiber | Acetone/acetic acid | CA | IBU/gliadin | Tuning drug release | [78] |
Core–shell fiber | Acetone/acetate acid | CA | FA/gliadin | Accurately tune drug release (zero-order) from nanofiber | [43] |
Core–shell fiber | Air blowing | PAN | TPU | Air-blowing assisted electrospinning can improve mass production | [79] |
Hollow fiber | Air blowing | PVP | Mineral oil | ||
Core–shell fiber | Ethanol | KET/EC | KET | High quality drug nanofiber depots for zero-order drug release | [80] |
A summary of the working performed on triaxial electrospun fibers in the last decade
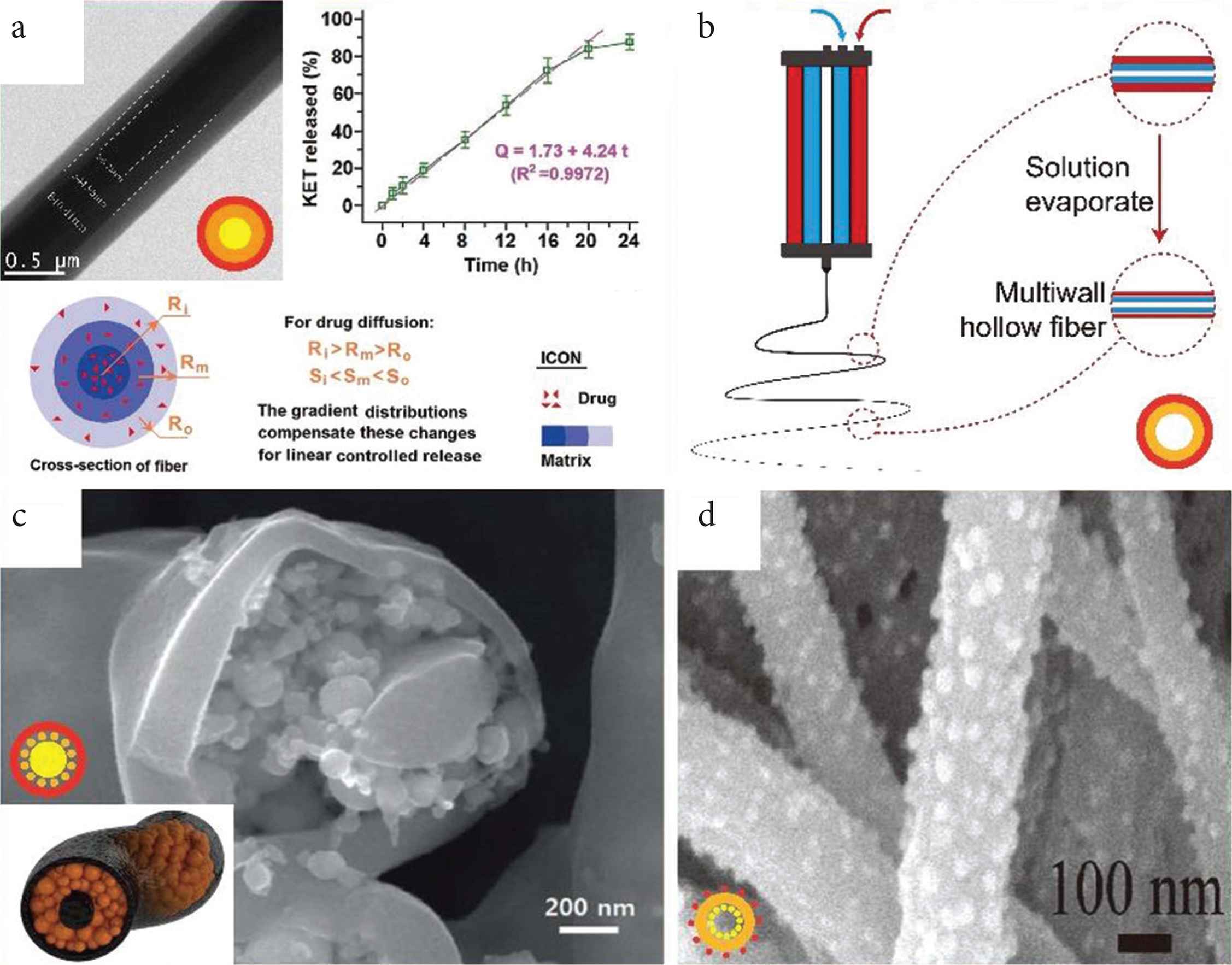
Selected three-layer structures prepared by triaxial electrospinning. (a) Triaxial nanofibers (TEM image) providing a linear drug release profile with a schematic explanation. (b) Diagram of preparing multiwall hollow structure fibers. (c) Tri-layer nanofiber with nanoparticles stored in medium layer (SEM image) and schematic diagram (inset). (d) Single-wall nanofiber decorated with nanoparticles inside and outside (SEM image).
Triaxial electrospinning can also be used to prepare core–shell and hollow structures. Using a pure solvent as the outer layer can protect the inner fluids preventing the solvent evaporating too fast and siding the formation the smooth fibers (Figure 3d). Furthermore, air can also be used as the shell in triaxial electrospinning as a role to increase the productivity of fiber by enhancing flow rate (Figure 3e). Hollow fiber and multiwall hollow fiber prepared by triaxial electrospinning have also been reported as Figure (3c and 3f) and Zanjani et al. [4].
The utilize of mediate layer in triaxial fibers has been explored for many purposes. For example, the middle layer can be utilized as a restricted zone to realize molecule self-assemble into ultrafine nanoparticles [60]; a “nanowire in nanotube” structure can be prepared by removing the middle layer [61], and the middle layer can also act as a reinforcing phase for enhanced mechanical properties [62]. With further treatment including removal, carbonization, and modified, various morphology and structure will be obtained. For instance, Lee et al. combined triaxial electrospinning with carbonization to prepare a C–Si–C tri-layer nanofiber formulation, depicted in shown as Figure 5c. This has a very high specific capacity at a high current rate and potential in lithium-ion batteries [63]. Chen et al. [64] remove the mineral oil core of the core–shell fiber and decorated the resultant hollow fiber with nanoparticles (as Figure 5d), which gain led to used for good performance in Li-ion batteries.
The further development of multifluid electrospinning lies in two aspects. One is the creation of complex nanofibers on a large scale. Different from monoaxial electrospinning, very limited researches have been reported on the scale-up production of complex structural nanofibers using multifluid electrospinning, although the scaling up of monoaxial electrospinning has been reported for many years, particularly the needleless electrospinning, which is low-cost and high-efficient [81]. The other aspect is the reasonable design of multi-chamber spinnerets for implementing the corresponding multifluid electrospinning processes. This is not an easy thing because of the mutual influences of multiple factors. With the double-chamber side-by-side spinneret as an example, the repulsion of two working fluids with the same charges, their contact surface area at the co-exit, and their flow rates will co-exist on the behaviors of working fluids under the electrical fields, and in turn the integrity of the final Janus fibers.
2.4. Other Multifluid Electrospinning Processes
Core–shell and Janus structures have been most widely researched in multifluid electrospinning. However, other multifluid electrospinning can also be used to develop more complex, for example, multichannel nanofibers were prepared by two to five working fluids packaged through sheath solution [82,83]; high-quality Janus fiber was fabricated by surrounding solvent by using a complex structure spinneret shown as Figure 4A(b and d) [55].
A four-layer fiber material has been prepared from a four-needle multiaxial electrospinning process. Labbaf et al. use Polyethylene Glycol (PEG), Poly(lactic-co-glycolic Acid) (PLGA), polycaprolactone (PCL) and polymethylsilsesquioxane as the outermost layer, second outermost layer, second innermost layer and innermost layer, respectively [30]. It is clear that the more layers bring more possible complex structures just like coaxial electrospinning and triaxial electrospinning.
3. BIO-APPLICATIONS
Owing to its advantages in developing nanofibers through a “one-step” process, the technology has been applied in various biological fields including drug release, tissue engineering, biosensor, wound dressing, theranostics and functional textile. Some of the functions which have been realized using electrospun fibers are depicted schematically in Figure 6.
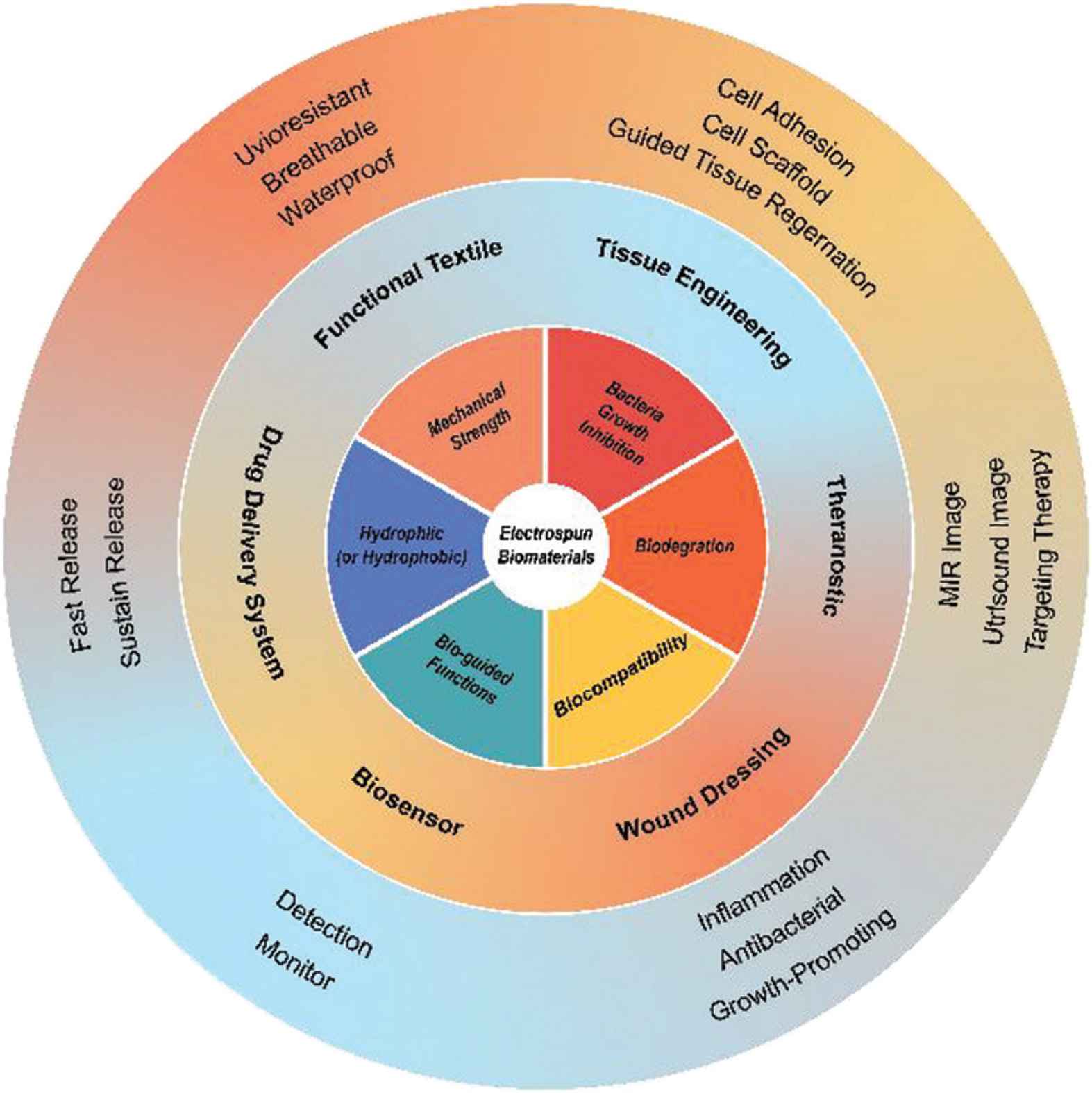
Schematic of the potential of electrospun nanofibers for use in biomedicine.
3.1. Tissue Engineering
3.1.1. Cell scaffolds
Scaffolds play a crucial part in tissue engineering, aiding cell adhesion, growth, and proliferation. Effective scaffolds in tissue engineering: (1) be biocompatible and allow cells to adhere to them for growth; (2) have appropriate mechanical strength; (3) mimic the native extracellular matrix; (4) provide appropriate functionality at the implantation site; (5) possible sufficient durability.
Hydroxyapatite (HA) is extensively used in preparing bone tissue scaffolds. By using a mixture of hydroxyapatite and Tussah Silk Fibroin (TSF) as a core solution and pure TSF as the shell solution, researchers have used multifluid electrospinning to prepare scaffolds for use in bone tissue engineering. Compared with pure TSF scaffolds, the core–shell structure improves both strength of extension, and biocompatibility of scaffold [84].
Multifluid electrospinning processes have also been used to prepare effective vascular tissue scaffolds [86]. However, in uniaxial electrospinning process, there is a trade-off among different functions within one fiber. With using coaxial electrospinning, core–shell fibers are prepared with better mechanical property than other fibers shown in Figure 7. A tubular vascular tissue engineering scaffold comprising PCL/collagen core–shell nanofibers was prepared by using coaxial electrospinning and found to have good biocompatibility and cell affinity [87]. Core–shell electrospun scaffolds with suitable mechanical properties, good hydrophilicity, the ability to promote osteoblast maturation and antibacterial properties were prepared by De-Paula et al. [88]. Coimbra et al. used PCL and synthetic gel-methacrylate (GelMA) in coaxial electrospinning to prepare a core-shell nanofiber network for vascular tissue regeneration. The content of gelatine influences the size distribution of the fibers and their hydrophilicity [89].
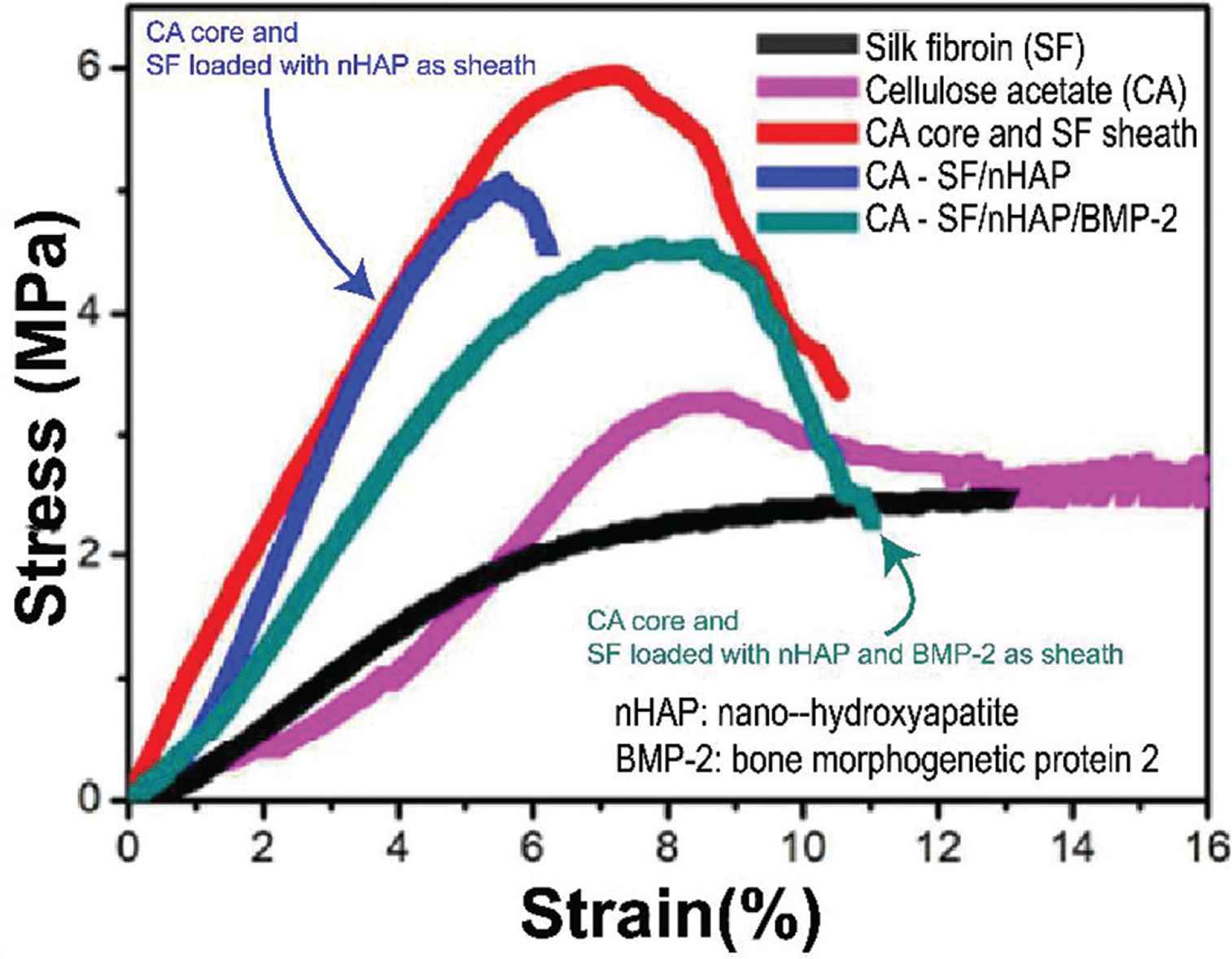
Multifluid electrospinning used for improving the mechanical strength and functions of core–shell fibers [85].
3.1.2. Cell adhesion
Since the expending of raw materials that can be used in electrospinning, various applications have been developed, such as peptide, protein [90], which benefit for developing the applications of electrospinning within biofield.
Biomaterials for cell adhesion must have the following properties: (1) Biocompatible materials, which allow cell can alive on and is safe to implant in the body or put on the skin, are necessary, such as gelatin, PCL, chitosan, Polylactic Acid (PLA) and so on [91–93]; (2) cell should has interaction with the biomaterial, which can improve the adhesion by incorporating specific materials, such as ZnO [94,95], peptides [96] (Arg-Gly-Asp [97], Arg-Glu-Asp-Val [98], CREDVW and CAGW [99]), protein [100] (silk fibroin [101], collagen [102]); (3) some cell adhesion biomaterials should possess the ability in improving cell proliferation and guiding their direction [103]. A highly anisotropic array film compose of Janus nanoribbons may provide a new strategy in guiding cell proliferation [104,105].
With the using of multifluid electrospinning, various functional molecules can be incorporated into cell adhesion biomaterials. Shalumon et al. [106] prepared a kind of antibacterial core–shell nanofiber membrane which can be used for tendon adhesion with long-time anti-bacterial. Besides the selecting of various materials, some other reasons also affect cell adhesion, such as texture. Hughes-Brittain et al. [107] explored the influence of endothelial cell adhesion on the surface texture electrospun fibers, which means not only materials, but also texture on the fiber can also affect cell adhesion.
3.1.3. Bio-regeneration
Guided Tissue Regeneration (GTR) and Guided Bone Regeneration (GBR) membrane are two major present foci in bio-regeneration. Multiple functions are needed to realize effective GTR and GBR membranes, such as drug release, durability, and guiding function. The traditional uniaxial electrospinning process can potentially might able to integrate multiple functions in a monolithic fiber with further treatments, but multifluid electrospinning can implement simpler in one-step. Tang et al. [108] used a coaxial process to produce a GTR membrane from PLGA and hydroxyapatite as the core and collagen and amoxicillin as the shell, and prepared core–shell fiber that performs well. Moreover, the effect of each function needs to be controlled. The initial burst release as a difficulty of the drug release process needs to be solved. In order to inhibit the production of bacteria on a scaffold, the drug release time needs to be extended [109]. We need the GTR to remain intact in the body for more than two months to ensure complete tissue repair [108]. Uniaxial electrospinning processes used to mix some substances into monolithic fiber. By using multifluid electrospinning processes, different layers perform their functions and work together.
Infection is a major for the failure of GBR membranes, and thus an extended period of drug release is required to prevent this and aid successful bone regeneration. Wang et al. [110] prepared electrospun PCL/gelatin core–shell nanofibers loaded with metronidazole in the core and nano-hydroxyapatite in the shell which prolonged the antibacterial efficacy and lowered the cytotoxicity of metronidazole. Xie et al. prepared core–shell fibers to control gene transfer to human periodontal ligament stem cells. This core–shell scaffold has an excellent gene release behavior and exhibited a prolonged-expression time [111].
3.2. Drug Delivery System
Along with the modern medicine booming, modern synthetic drugs are also present exponential growth, but most of the synthetic drug exists in the crystalline, poorly soluble in water that is substantially limited in application. Electrospinning appeared as a convenient, rapid prototyping technology, which can solidify working fluid in a very short period. It takes a uniform water-insoluble drug dissolve suitable solvent as the working fluid, which removes the solvent by rapid evaporation in the electric field, and fixes the drug to an amorphous state. In one recent study, Wang et al. used hydrophilic hydroxypropyl methylcellulose as the fiber-forming matrix polymer and an insoluble model drug Ferulic Acid (FA). Modified coaxial electrospinning was applied to produce nanofibers, which were found to release the embedded FA quickly. And X-ray diffraction result shows that FA was dispersed in nanofibers in an amorphous form [112].
However, in the process of drug release there typically an initial burst release and later tailing off when working with monolithic fibers from unaxial electrospinning. An appropriate burst release process will increase drug concentration to effective drug concentration in a short time, which requires precise control and constraint on drug release. Han and Steckl [67] observed the phenomenon of initial explosive release and later sustain release by using two dyes as release models through triaxial electrospinning. However, an initial burst if release can result in overly high drug concentrations which cause toxicity to the body; late trailing may lead to drug concentrations that are insufficient to produce the desired therapeutic effect. Wither initial burst can be control or not is important.
Using triaxial electrospinning technology, Yu et al. set different concentrations of Ketoprofen (KET) in the inner, middle, and outer working fluids, respectively. Furthermore, they took Ethyl Cellulose (EC) as the matrix to realize a zero-order drug release profile, with the release time exceeding 20 h as Figure 5a [69]. This team also used the same technology to encapsulate FA, an insoluble drug, in the CA, to achieve zero-order drug release over 36 h [76]. The use of the shell can also alleviate the initial burst [66]. Yang et al. [78] obtained the CA/ibuprofen-gliadin core–shell fiber by using modified triaxial electrospinning, which eliminated the initial burst and prolonged the drug release time. Later, this team explored the relationship between the thickness of CA shell and drug release through modified triaxial electrospinning [43].
In addition, it can often be desirable to deliver multiple drugs at the same time. One route to do this is to load multiple drugs in the same fiber formulation. Jouybari et al. [77] prepared three degradable three-layer fibers that can simultaneously control the release of three drugs through triaxial electrospinning, which can be used for treating breast cancer in vitro. Kim and Kim [66] prepared PLGA/Poly(DL-Lactic Acid) (PDLLA) biodegradable triple-layered capsules for the release of taxol and doxorucin by triaxial electrospraying [66]. A more intuitive approach is to use side-by-side fibers to release two drugs. By using Polyvinyl Pyrrolidone (PVP) K60 and EC as substrates, Yu et al. [113] prepared parallel fibers that controlled the different release rate of KET.
3.3. Wound Dressing
Biocompatibility and antibacterial properties are key for effective wound dressings. Various antibacterial materials have been applied using electrospun nanofibers to generate wound dressing materials, such as Ag Nanoparticles (AgNPs) [114,115], honey [116], vitamin E [117], broad-spectrum antimicrobial (such as trimethoxysilylpropyl octadecyldimethyl ammonium chloride (QAS), ciprofloxacin, tetracycline hydrochloride and simvastatin) [118–120], peptides [121] and so on. However, the majority of studies have been undertaken using single-fluid electrospinning, which is problematic for fibers from single-fluid electrospinning cannot draw drug release profiles very well compared with multifluid electrospinning.
Multifluid Electrospinning could diminution wound dressing to nanoscale into fiber membranes and achieve good antibacterial properties and anti-inflammatory properties. Kalwar et al. [122] prepared a core–shell fiber membrane comprising PCL@chitosan nanofibers incorporating AgNPs, and found them to have good antibacterial properties. By using coaxial electrospinning, Heydari et al. [119] obtained an antibacterial and healing promotion core–shell membranes by loading simvastatin in the core and ciprofloxacin in the shell. Wen et al. [123] also prepared a dual-functional core–shell electrospun mat with the ability to accurately control the release of anti-inflammatory and anti-bacterial agents.
Nanofibers from multifluid electrospinning can also combine other functions, such as mechanical property, healing-promoting, biodegradable. Zahedi et al. [124] prepared core–shell fibers with a core containing Polyethylene Oxide (PEO)/Aloe vera extract and a shell comprising PCL/chitosan/keratin, which has great mechanical strength and can be used for wound healing. Wei et al. [125] use AgNPs and vitamin A palmitate as an antibacterial agent and healing-promoting drug in develop core–shell fibers. To overcome problems with wound healing in patients with type-2 diabetes, Augustine et al. [126] used Connective Tissue Growth Factor (CTGF) in the preparation of membranes composed of nanofibers with a CTGF-Polyvinyl Alcohol (PVA) core and PLA shell, and these membranes were found to have excellent potential in cell proliferation, migration and angiogenesis. By utilizing the coaxial electrospinning process, Fang et al. prepared biodegradable nanofibers, which comprising poly(γ-glutamic acid) as the core and PLA as the shell, with more than 90% re-epithelialization [127].
3.4. Biosensor
Electrospun fibers can be used in sensors for gas detection, such as ethanol [41], acetone [40], H2 [128], etc. However, when the sensor embedded in the body, it is a different environment and different substances needed to detect, such as dopamine [129], aflatoxin B1 [130], glucose [131,132], 25-hydroxy vitamin‑D3 [133], urea [134], etc.
To improving sensitive and selective sensors, many tools have been incorporated in or loaded on electrospun fibers and used to detect singles, such as metal catalysts [129], inorganic compounds [131,135], enzymes [136,137], antibodies [130], etc. Because of the very large specific surface area of electrospun nanofibers, electrospun biosensors have been found to be much more sensitive electrochemical than conventional sensors. Using multi-fluid electrospinning processes, hollow porous nanofibers can be prepared (see Figure 8a–8c) to construct heterogeneous junctions between the shell and the nanoparticle formed on shell porous. shown as Figure 8e, which is conducive to the transformation of gas signals and electrical signals. From Figure 8d, it is clear that Pd-porous WO3 Nanotubes (NTs) performs better than porous WO3 NTs [128].
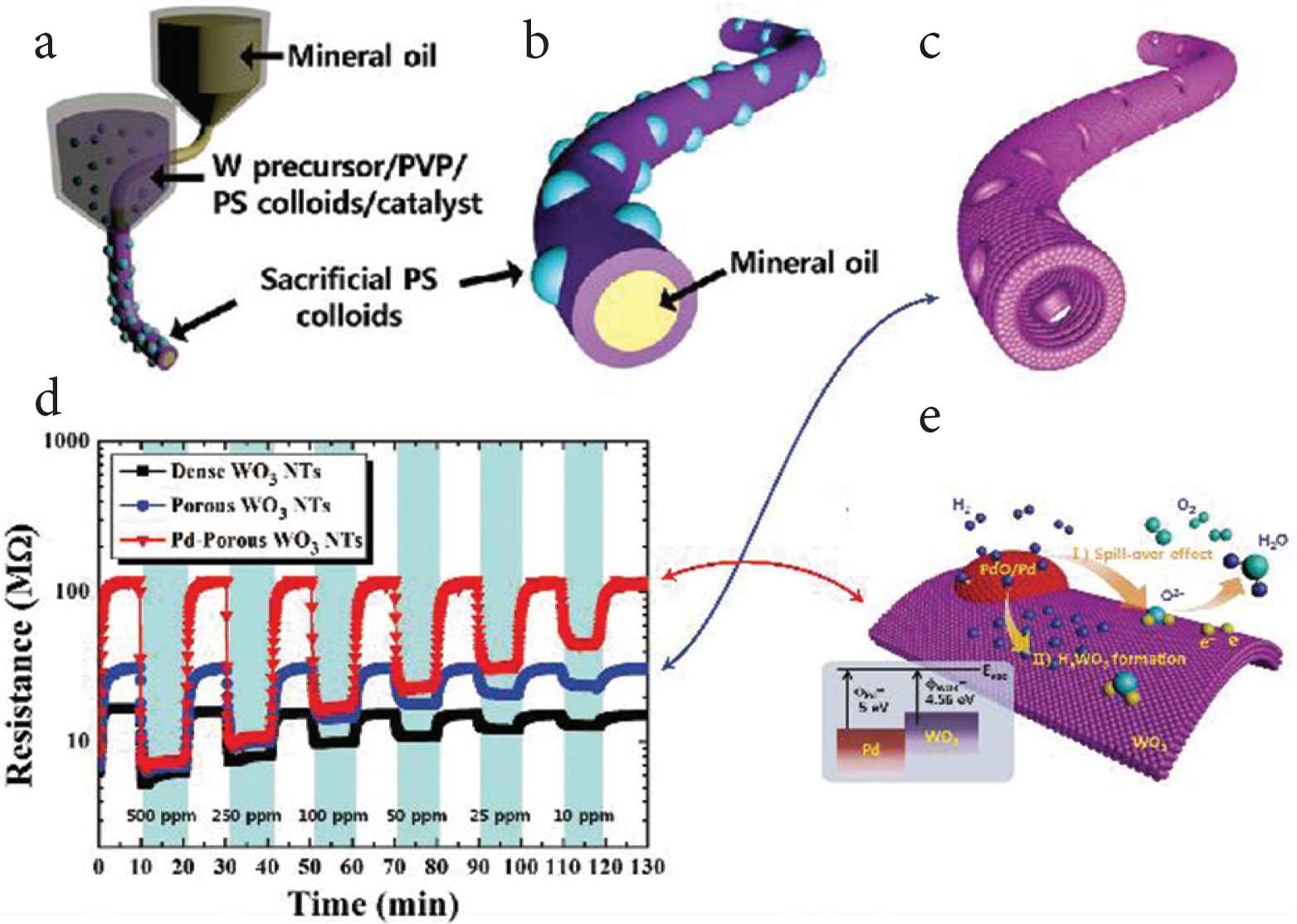
Core–shell nanofibers used for constructing heterojunction as a sensor. (a–c) WO3 NTs with multiple pores was prepared by coaxial electrospinning. (d) Dynamic resistance changes in detecting H2 using different materials: dense WO3 NTs, porous WO3 NTs, and Pd-porous WO3 NTs. (e) A schematic diagram of H2 sensing using Pd–porous WO3 NTs.
There are also reports of biosensor from coaxial electrospinning being used in vivo. Wang et al. prepared an implantable biosensor incorporating Pt-Glucose Oxidase (GOD) in order to detect glucose. The biosensor coated with core-shell fiber membranes, which compose 6 wt% polyurethane in core and 10 wt% gelatin in the shell, was immersed in phosphate buffer for 12 weeks also remain fiber morphology [138]. Furthermore, compared with core–shell heterogeneous [139], the Janus fibers can be used to construct heterogeneous junctions as well and load different tools to detect different substances. By utilizing heterogeneous junctions that play an important role in many fields [140], multifluid electrospinning processes can involve other fields easily.
3.5. Theranostics
Theranostics, which can diagnose and treat simultaneously, comprising an emerging field in biotechnology. It is one area where multifluid electrospinning could have great potential, because of its inherent ability to introduce multiple functions into nanomaterials. Signals, which can be detected by advanced technology or eyes or feelings, are essential characteristics that theranostic biomaterials should possess.
By using coaxial electrospinning, Jin et al. prepared core–shell nanofibers containing gadolinium (III) Diethylenetriaminepentaacetate [Gd(DTPA)] hydrate Gd(DTPA) as a contrast agent core and Eudragit S100 as a shell to allow the targeting of drug release. This core–shell nanofiber can be used for magnetic resonance imaging of the colon ex vivo [141]. This team also research on the simultaneous imaging and sustained drug release over 12–29 h at pH 7.4 by using core–shell nanofibers [142].
3.6. Functional Textiles
Functional textiles based on electrospun nanofibers possess great potential. Many functions can be incorporated into membranes through single fluid electrospinning, such as waterproofing [143], antibacterial [144] and conductive [145] properties. Bin et al. have reported many studies on electrospun functional textiles for water-transport [146], ultraviolet resistance, waterproofing [147], waterproof-breathable [148], and air filters used for protect from PM2.5 [149].
We believe that multifluid electrospinning can perform better than monoaxial electrospinning in preparing multifunctional textiles, and is likely to lead to nanofiber membranes with more functions and better performance. Even so, problems remain to be overcome, such as scaleup. Scaling up monoaxial electrospinning has been explored for many years, and various solutions have been proposed [81]. However, relatively few reports discuss coaxial electrospinning scale-up [150], because of the difficulty of handling multiple fluids and ensuring that the desired multi-compartment products are generated.
4. CONCLUSION AND PROSPECTS
As a facile, low-cost, and flexible method to produce nanofibers, electrospinning has been explored in various bio-fields and the products have been found to have very good performance in many cases. We summarize here a range of different multifluid electrospinning processes, discussing some of the key parameters which must be considered in implementing an effective and reproducible process. Biomaterials composed of nanofibers prepared by multifluid electrospinning have been introduced, and selected studies highlighted to show the enhanced properties which can be achieved compared to the monolithic fibers from single-fluid spinning. Multifluid electrospinning processes can produce materials with many functions and structures that cannot be realized when working with a single solution. Challenges, such as the difficulty in scaling up multifluid electrospinning and structural spinnerets, have also been introduced. It is clear from the limited publications about multifluid electrospinning that the electrospun complicated nanostructures always showed a better functional performance than the corresponding monolithic nanofibers [151]. The reasons are obvious that multiple-chamber nanostructures are able to provide more opportunities for tailoring the components, compositions, and ingredient spatial distributions within the nanofibers, and thus act as a more powerful platform than the traditional blending electrospinning for endowing the electrospun nano products the desired functional performances [152,153]. In the authors’ view multifluid electrospinning is likely to attract increasing attention owing to the exceptional performance that can be realized.
CONFLICTS OF INTEREST
The authors declare they have no conflicts of interest.
REFERENCES
Cite This Article
TY - JOUR AU - Menglong Wang AU - Deng-Guang Yu AU - Xiaoyan Li AU - Gareth R Williams PY - 2020 DA - 2020/06/08 TI - The Development and Bio-applications of Multifluid Electrospinning JO - Materials Highlights SP - 1 EP - 13 VL - 1 IS - 1-2 SN - 2666-4933 UR - https://doi.org/10.2991/mathi.k.200521.001 DO - https://doi.org/10.2991/mathi.k.200521.001 ID - Wang2020 ER -